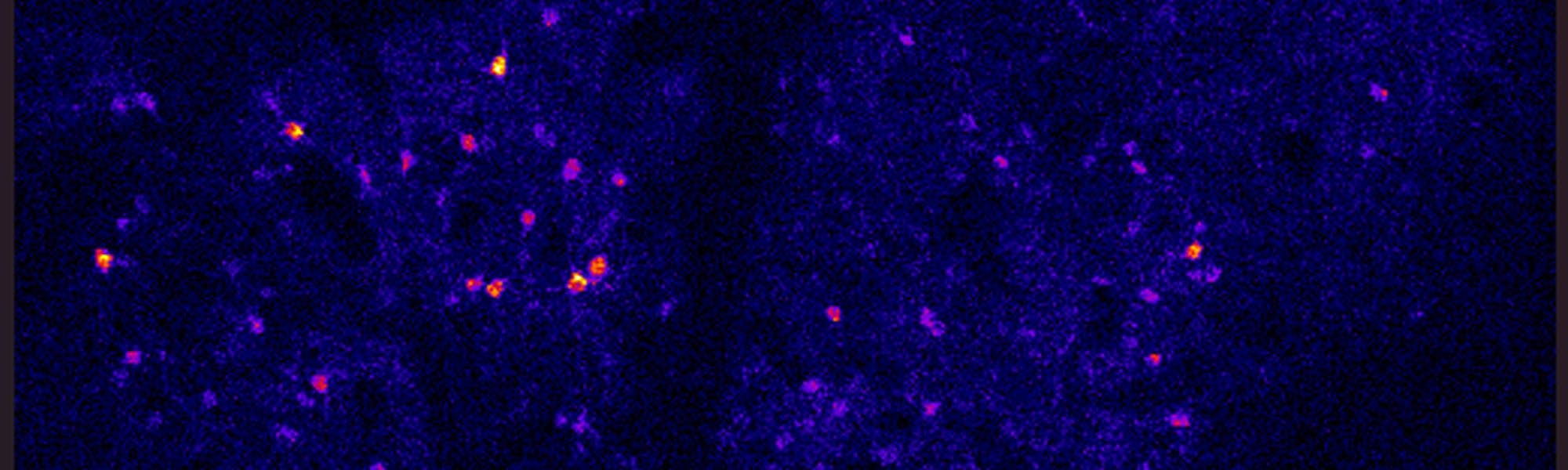
In vivo electrophysiology: benefits, weakness, and alternative solutions
by Dr. Francesca Chaloner (Samuel Cooke Lab, King’s College London)
Introduction
Electrical activity of neurons is a primary mechanism of information processing in the brain. The electrical events produced at single synapses, by individual neurons or by populations of either produce electrical signals which can be recorded with high fidelity using electrophysiology across a variety of scales. Depending on the questions that are being asked about brain function, recording select scales or types of signals may be most informative, and recording each requires a different method. For instance, the gold standard method to monitor activity at individual synapses is ex-vivo whole cell patch clamp electrophysiology, typically using a blunt, fire-polished, glass pipette recording electrode. This method not only allows recording of on-going miniature, spontaneous or evoked synaptic events, as well as action potentials, but it also enables experimental constraint of voltage across the neuronal membrane, thereby providing deep insight into the biophysical mechanisms at play in each type of electrical event, whether it be a synaptic or action potential. Moreover, ex-vivo techniques greatly aid cell-specific targeting for recording and visualization of specific synaptic inputs for stimulation. However, there are obvious limitations to ex-vivo electrophysiology, notably that it does not allow for the study of the brain as it subserves its primary purposes of sensation, perception, cognition, and action. There are now several laboratories that have taken on the heroic endeavour of performing these intracellular recordings in-vivo and, on occasion, in the awake, behaving animal. However, these experimental approaches have low yields and require many years of training. Another key limitation of intracellular or whole cell recording methods is that they typically limit the experimenter to recording from just a couple of cells at once. Recordings of the population dynamics of hundreds of cells within an intact system can be achieved with extracellular electrophysiology. Different types of in-vivo electrophysiological techniques allow for recording of action potentials, or spikes, produced by single neurons, or larger scale signals that reflect summated synaptic activity or action potentials recorded from many neurons at once. I will now discuss these methods below.
Most common forms of in vivo electrophysiology
Silicone laminar probes
Laminar probes are used to record single units (action potentials) across multiple cell layers. They contain many electrodes along a shank and require hardware to record at fast acquisition rates. Fast acquisition rates (40kHz; data point collected every 0.025 ms) are required to resolve the action potential waveforms (~1ms) generated by multiple neurons.
Local field potentials
Local field potentials (LFPs) can be recorded using chronically implanted tungsten electrodes. This is a signal which originates from ~250 microns around the electrode (Katzner et al., 2009), therefore reflecting relatively localized neural activity. The LFP predominantly reflects summed synaptic activity and membrane voltage fluctuations as these slower events are more likely to summate than fast action potentials. However, other neural processes (e.g action potentials, glial activity etc) contribute partially to the LFP signal. The contribution of action potentials is notable if these events are evoked by a super-threshold electrical stimulation of afferent fibres that tightly synchronizes action potentials produced by post-synaptic neurons. This so-called ‘population spike’ is most commonly described in the hippocampus, where there is an advantageous laminar arrangement comprising a single layer of excitatory neurons and neatly arranged dendritic subfields on either side, as well as neatly defined feed-forward afferent pathways, allowing for relatively easy interpretation of evoked local field potential responses as either an early synaptic slope or a later population spike signal which typically occur in opposing directions.
Intercranial EEG
Electrical signals can be recorded from the surface of the brain using screws. Intracranial EEG reflects activity from broader spatial locations. Activity from distant and local brain regions will contribute to the signal recorded from the surface of the brain. Again, summed synaptic activity is the largest contributing factor to this signal but other neuronal electrical processes will contribute to the EEG signal. This signal similar to human EEG, albeit slightly less attenuated as it is recorded from the surface of the brain rather than the surface of the skin around the skull.
Type of data recorded using in-vivo electrophysiology
Stimulus-evoked potentials
Sensory-evoked responses occur after presentation of a sensory stimulus, for example visual evoked potentials (Figure 1). Evoked potentials are observed in the signal time-series when averaging over many trials of sensory stimuli. They predominantly reflect summed synaptic events in symmetrically aligned neuronal populations across cortical laminae in response to sensory stimuli. These evoked potentials can be observed in mouse LFP recordings and human EEG recordings, where by necessity they are recorded from neocortex.
Event-related potentials may also be evoked by direct stimulation. Non-invasive methods for evoking include transcranial magnetic stimulation (TMS). As described above, potentials may also be evoked by electrical stimulation within a defined afferent pathway, in which case there may be a population spike component to the evoked potential in addition to summated synaptic events. A classic experimental example of the use of this approach are monosynaptically evoked field potentials in the hippocampus, which were key to the discovery of various forms of plasticity in-vivo, including long-term potentiation (LTP).
Event-related potentials may also be evoked by direct stimulation. Non-invasive methods for evoking include transcranial magnetic stimulation (TMS). As described above, potentials may also be evoked by electrical stimulation within a defined afferent pathway, in which case there may be a population spike component to the evoked potential in addition to summated synaptic events. A classic experimental example of the use of this approach are monosynaptically evoked field potentials in the hippocampus, which were key to the discovery of various forms of plasticity in-vivo, including long-term potentiation (LTP).
The Frequency domain
Brain activity follows specific timing regimes, and these can be observed as phasic activity recorded in local field potentials and EEG (Buzsáki, Anastassiou and Koch, 2012; Cohen, 2017). Frequency analysis can be performed to determine the frequency components that make up an electrophysiological signal. Different oscillations occur during different processing modes and are often reflective of the influence of various forms of inhibition and/or neuromodulation. Higher-frequency states, like gamma (30-80 Hz) and Beta (13-30 Hz) are associated with active processing, whereas lower-frequency states, like alpha (8-12 Hz) and Delta (0.5-4 Hz) are associated with quiet wakefulness and sleep, respectively (Alitto and Dan, 2010).
Action potentials
Spiking output of neurons (action potentials) can be recorded from multiple cells in many layers at fast acquisition speeds (40 kHz compared to 1 kHz for EEG/ LFP). Action potentials can be assigned to individual neurons via ‘spike sorting’ (Rossant et al., 2016) to investigate changes in firing of neurons across different layers and different timescales in response to a variety of stimuli and during a variety of behaviours. This technique has been critical in identifying a wide range of receptive fields (those regions of exterior space or stimulus properties that selectively drive activity in a particular neuron) for individual neurons in various regions of the brain. Such receptive fields include orientation-selective ‘simple’ cells in primary visual cortex, ‘place’ cells in hippocampus, ‘grid’ cells in entorhinal cortex and ‘mirror’ cells in premotor areas of the brain (Hubel and Wiesel, 1962; O’Keefe and Nadel, 1979; Gallese et al., 1996; Moser and Moser, 1998). None of these key computational units of brain function could have been identified without performing experiments in the living animal and, in most cases, the awake, behaving animal. Thus, extracellular recording of spikes is a key method for understanding the role different regions of the brain in perception, cognition and action.
Advantages of in vivo electrophysiology
- Record signals from deeper within the brain than calcium imaging
- High temporal resolution of the activity of neurons (acquisition occurs from from 1 kHz to 40 kHz)
- Less invasive than cranial window implantation for calcium imaging
- Translatable signal – similar to EEG in humans
- Recording from multiple brain regions simultaneously through implantation of electrodes in multiple regions
- Chronic recordings over days and months
- Recordings can be performed in freely-moving animals
- LFP and EEG are simple and relatively cheap to implement; especially when using open-source systems (e.g. OpenEphys) and homemade electrodes for LFP (tungsten wire / gold pins).
Weaknesses of in vivo electrophysiology
- Laminar probes are expensive and use in chronic longitudinal recordings prevents reuse of the electrode. Acute recordings with laminar probes allow reuse but limits analysis to single sessions rather than longitudinal recordings and usually requires very stable head-fixation.
- Inability to localize the cell type(s) which are generating the signal.
- Cannot directly record inhibitory and excitatory synaptic currents – the extracellular signal (LFP/EEG) reflects both excitatory and inhibitory synaptic currents and many other electrical events in the brain.
- Spiking can only be attributed to fast spiking (putative parvalbumin-positive neurons) and regular spiking (putative excitatory neurons) – the myriad of other cell types are lumped into these two categories and cannot be deciphered without other complementary techniques.
Alternative solutions to address the weaknesses of in-vivo electrophysiology
Localization of action potentials to specific cell types
Mouse lines expressing Cre or other recombinases in specific cell types are common tools in neuroscience. Using these lines, optogenetic actuators can be expressed to activate specific cell sub-types with light. Optogenetic activation of a specific cell type while laminar probes are implanted allows allocation of specific spike waveforms to a cell type of interest; activating a cell with light triggers an action potential which is directly recorded on the laminar probe. Thus this specific spike waveform can now be confirmed to be from that cell type of interest (Lima et al., 2009).
In-vivo electrophysiology and ex-vivo electrophysiology
To provide a more complete picture of the changes in the brain in-vivo
electrophysiology can be used in concert with ex-vivo electrophysiology (Antoine et al., 2019). Thus, any changes observed in-vivo in the evoked potentials, frequency domain and spiking can be attributed to intrinsic properties, such as firing rate or action potential threshold, of individual neurons. Or the in-vivo results may reflect changes in the excitatory or inhibitory inputs these cells receive.
Conclusion
To conclude, in-vivo electrophysiology is a powerful technique which provides a highly temporally precise readout of the activity of the brain in multiple regions and across many depths in awake behaving animals. This approach has proved transformative in understanding the computational role of the brain in sensation, perception, cognition and action. Advanced contemporary biological tools (e.g. chemogenetics, optogenetics, disease models) used in many aspects of neuroscience research can now be used in combination with in-vivo electrophysiology to provide a greater understanding of cells and circuits in the brain and dysfunction in disease. However, multiple other techniques (e.g. ex-vivo electrophysiology, calcium imaging) may need to be combined with in-vivo electrophysiology to combat the weaknesses associated with this technique.
References
Alitto, H.J. and Dan, Y. (2010) “Function of inhibition in visual cortical processing.,” Current Opinion in Neurobiology, 20(3), pp. 340–346. doi:10.1016/j.conb.2010.02.012.
Antoine, M.W. et al. (2019) “Increased Excitation-Inhibition Ratio Stabilizes Synapse and Circuit Excitability in Four Autism Mouse Models.,” Neuron, 101(4), pp. 648-661.e4. doi:10.1016/j.neuron.2018.12.026.
Bliss, T.V. and Lomo, T. (1973) “Long-lasting potentiation of synaptic transmission in the dentate area of the anaesthetized rabbit following stimulation of the perforant path.,” The Journal of Physiology, 232(2), pp. 331–356. doi:10.1113/jphysiol.1973.sp010273.
Buzsáki, G., Anastassiou, C.A. and Koch, C. (2012) “The origin of extracellular fields and currents--EEG, ECoG, LFP and spikes.,” Nature Reviews. Neuroscience, 13(6), pp. 407–420. doi:10.1038/nrn3241.
Cohen, M.X. (2017) “Where does EEG come from and what does it mean?,” Trends in Neurosciences, 40(4), pp. 208–218. doi:10.1016/j.tins.2017.02.004.
Gallese, V. et al. (1996) “Action recognition in the premotor cortex.,” Brain: A Journal of Neurology, 119 ( Pt 2), pp. 593–609. doi:10.1093/brain/119.2.593.
Hubel, D.H. and Wiesel, T.N. (1962) “Receptive fields, binocular interaction and functional architecture in the cat’s visual cortex.,” The Journal of Physiology, 160, pp. 106–154. doi:10.1113/jphysiol.1962.sp006837.
Katzner, S. et al. (2009) “Local origin of field potentials in visual cortex.,” Neuron, 61(1), pp. 35–41. doi:10.1016/j.neuron.2008.11.016.
Lima, S.Q. et al. (2009) “PINP: a new method of tagging neuronal populations for identification during in vivo electrophysiological recording.,” Plos One, 4(7), p. e6099. doi:10.1371/journal.pone.0006099.
Moser, M.B. and Moser, E.I. (1998) “Distributed encoding and retrieval of spatial memory in the hippocampus.,” The Journal of Neuroscience, 18(18), pp. 7535–7542. doi:10.1523/JNEUROSCI.18-18-07535.1998.