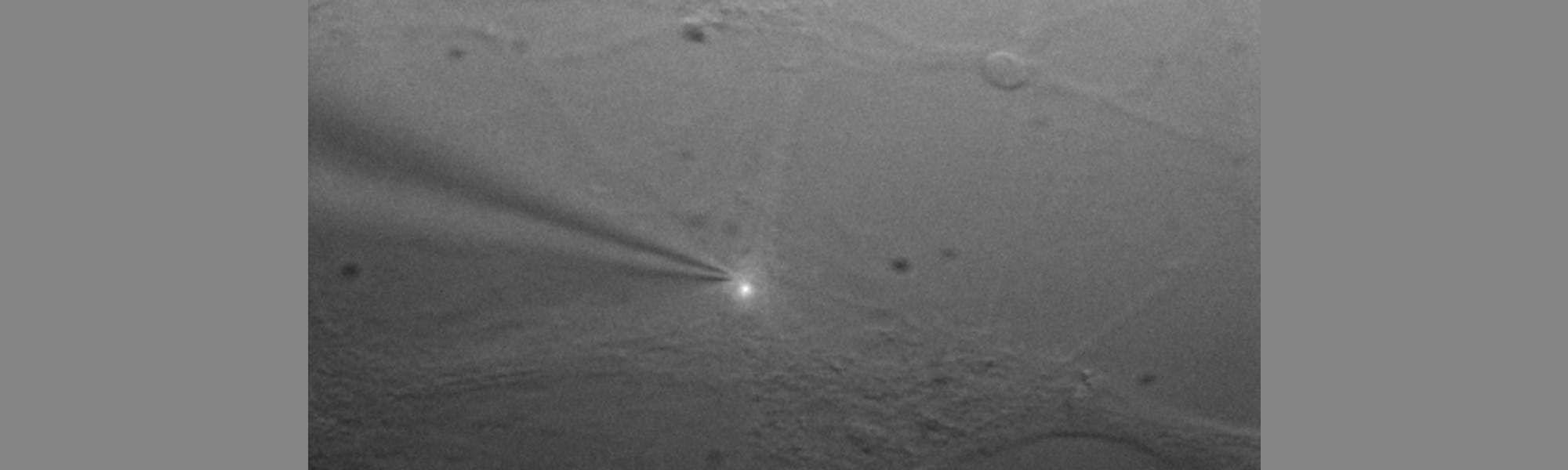
Planning and optimising an optogenetics experiment
Daman Rathore, UCL
The emergence of optogenetics has resulted in great advancement in the way neuroscientists can investigate neuronal connectivity and communication within brain networks. Until recently, the invasiveness and time-consuming nature of patch clamp electrophysiology has limited our understanding of complex signalling within networks. The development of optogenetic stimulation now allows precise modulation of population activity in the forms of excitation, inhibition or G-protein-coupled receptor (GPCR) activation. Here we discuss important factors to consider when planning an optogenetics experiment. In particular, we focus on steps to optimise experimental parameters and allow efficient characterisation of neuronal signalling in different experimental environments.
The opsin: Input to output
The desired effect
Since the initial characterisation of the first Channelrhodopsin (ChR), a large number of optogenetic tools— both ionotropic and metabotropic— have been developed, resulting in a vast array of instruments that vary greatly in their properties (Kim et al., 2017). Essential to experimental design is the selection of a tool that is able to elicit the desired effect; recent developments in the field have resulted in great flexibility in the tools themselves. The first application of optogenetics in dissociated hippocampal cultures led to the widespread understanding that Channelrhodopsin-2 (ChR2) was a highly effective ion channel in producing light-activatable neuronal excitation (Boyden et al., 2005). Since then, numerous different ChR variants have been discovered in nature and purposefully engineered through mutation to have desirable characteristics. One of these desired characteristics is the ability to produce fast, reliable inhibition of neuronal populations following photostimulation. Optical inhibition of neurons can be achieved through multiple diverse mechanisms, with some utilising anion-permeable channels (GtACR2; Messier et al., 2018), others utilising anion-permeable pumps (eNpHR3.0; Gradinaru et al., 2010), while some manipulate the outward movement of protons to result in hyperpolarisation (ArchT; Han et al., 2011). Lastly, optogenetics can now allow controlled modulation on a much slower time scale using chimeric OptoXRs. OptoXRs are photo-activatable GPCR-derived constructs that allow slow modulation of specific pathways, e.g. Gαs activation in β2 adrenoceptors (Kim et al., 2005).
The array of tools described above demonstrates the exceptional optical control neuroscientists can have over their cell of interest. The selection of a tool that generates the desired output is an essential first step in constructing an optogenetics experiment.
Producing the effect: Choosing the right excitation wavelength
A large evolution in optogenetics has come from the generation of ion channels that respond to red-shifted wavelength stimulation. This provides great flexibility, particularly in the case of in vivo experimentation, whereby deeper brain structures can be precisely targeted for stimulation, with a reduction in photon scattering compared to blue light illumination (Lin et al., 2013). Additionally, a useful feature of optogenetic experiments is their complementary nature when considering combination with imaging (Hochbaum et al., 2014). Many different methods exist to optically quantify both population and sub-cellular activity. Examples of these fluorescence optical reporters include Ca2+ indicators (Akerboom et al., 2013), voltage-sensitive dyes (Hochbaum et al., 2014) and neurotransmitter sensors that respond directly to neurotransmitter exocytosis (iGluSNFR; Marvin et al., 2013). An important consideration when planning optogenetics experiments is the spectral properties of the chosen proteins. A plethora of both red and blue light-excited optogenetic tools and optical reporters are now available that permit the imaging and optogenetics methods to occur in parallel. However, constructs with distinct spectral properties should be utilized to minimise any optogenetic stimulation due to optical reporter fluorophore excitation. Their combination can be tailored by selecting the appropriate excitation wavelengths and dichoric filters on the system to make the experiment feasible.
Maintaining control - channel sensitivity and kinetics
Optogenetic tools exhibit great diversity in many other properties besides excitation wavelength, including spatial distribution, kinetics and photocurrent. Consideration of these properties is essential when selecting optogenetic tools best suited to a specific application. For example, in the case of experimentation requiring reliable ChR-mediated generation of single action potentials, the kinetics are very important to consider. If the channel has slow off kinetics (e.g. CnChR2; Klapoetke et al., 2014), delivery of a single light pulse will result in the influx of cations for a substantial period of time, resulting in the generation of multiple action potentials with little control over firing frequency or count (Berndt et al., 2011). If controlled high-frequency optogenetic stimulation is required, a ChR with fast kinetics (e.g. Chronos; Klapoetke et al., 2014) should be selected to allow tight coupling between neuronal activity and light pulse delivery.
In recent years, optogenetic tools (for example, the excitatory CoChR) have been expressed in conjunction with different fusion proteins to target expression to cellular compartments, resulting in the generation of soma-specific constructs. Although systematic optimisation has resulted in the use of high photocurrent opsins, it’s important to select a light delivery and expression system that can achieve sufficient depolarisation (Shemesh et al., 2017). This is especially important in the case of somatic-ChR constructs as the total channel-containing surface of the membrane is largely reduced in comparison to untargeted constructs.
Where should it go?
Expression of the optogenetic actuator
Multiple techniques effectively modify neuronal expression of particular genes in different environments. Some techniques are feasible in all systems, whereas others are limited in their flexibility. Nonetheless, all offer considerable advantages over the other methods and it’s essential to consider the method of gene delivery in the context of the desired experiment.
Opsins can be introduced by viral transduction. Most commonly used viruses are adeno-associated viruses (AAVs) due to their high transduction efficiency (Kim et al., 2017). AAVs, unlike lentiviruses (Naldini et al., 1996), insert DNA into targeted cells without integration into the host genome, resulting in the increased potential for clinical translation (Naso et al., 2017). Moreover, when investigating functional connectivity, expression of optogenetic tools can be precisely targeted to presynaptic populations through the use of retrograde viruses e.g. rabies virus (Yamawaki et al., 2016). In the specific case of optogenetic stimulation, it’s important to consider the size of the region being targeted and the desired expression density/distribution within this region. This is achieved by refining the stereotaxic injection protocol by modifying parameters such as viral titre, injection volume and speed of injection (Cetin et al., 2007).
The methods used for expression induction in vivo carry the same benefits and limitations when used for ex vivo experimentation, however, experimental requirements will often differ. When considering in vitro optogenetic experiments, alternative benefits and limitations of transfection methods exist with additional methods available for gene expression manipulation. Lentiviruses are most commonly used to transduce a large proportion of cultured neurons. Although effective, a large quantity of AAV particles are required for efficient transduction and this consequently limits their usage in cultured samples. Due to the high transduction efficiency of lentiviruses, their application in some optogenetic experiments may be limited, for example, if attempting to measure monosynaptic responses using optogenetic stimulation with field LED illumination (see Section 4), low density expression of ChR constructs may be required. This is difficult to achieve using viral transduction, and various methods of plasmid transfection may be useful in this regard. Many of these methods (e.g. lipofection, calcium phosphate, magnetofection etc.) can effectively induce a high level of expression in a small proportion of cells, allowing selective stimulation of specific neurons without the requirement for complex/expensive light delivery systems (Karra and Dahm, 2010).
Lastly, inserting the gene encoding the optogenetic tool into the genome of the organism of interest is a highly effective method of expression for all experimental environments. A major advantage of this method is that it allows reliable, targeted and uniform expression in all cells of interest. This can be highly useful if experiments require only wild type mice and surgical facilities/consumables are limited. However, in the case of expression-induction in already transgenic animals, a large limitation comes with the cost of breeding; genomic expression in otherwise transgenic animals requires two separate colonies of the same background strain to be crossed with one another. Moreover, this limitation is particularly relevant in the case of Cre-dependent expression whereby co-expression of Cre-recombinase and a lox-site flanked gene allows targeted expression in specific cell types. These methods are extremely powerful in providing an extra dimension of flexibility when expressing optogenetic tools (Kim et al., 2017).
Choose the right promoter
When planning an experiment using optogenetics, it’s essential to consider which cells are being targeted for optogenetic modulation. In the case of ChR-mediated excitation, correct promoter selection is essential in ensuring measured responses are due to the intended cell being depolarised. Targeted expression of the protein of choice can be controlled by including an upstream, cell-type specific promoter in the DNA of the viral construct. Promoter-selective expression offers an exceptional advantage in the dissection of communication with optogenetic tools able to be targeted to broad classes of cells (Kim et al., 2017), such as neurons (Syn; Klapoetke et al., 2014) and astrocytes (GFAP; Perea et al., 2014). Additionally, many smaller populations of neurons can be targeted, e.g. excitatory pyramidal cells (CAMKII; Miyashita et al., 2013) and many different classes of interneurons, such as PV, CCK etc.(Chang et al., 2017, Mantoan Ritter et al., 2016). Finally, it’s crucial to select a promoter that produces an expression level sufficient to generate a large enough photocurrent to depolarise neurons to the threshold potential; this is especially important to consider in the case of two-photon excitation.
How to get it going: Light delivery
Depending on the complexity of the desired photostimulation paradigm, there are many light-delivery systems available to choose from. The first broad decision regarding stimulation is whether one-photon or two-photon stimulation is needed. The choice requires an assessment of whether the high additional cost of two-photon stimulation is justified by the exceptional increase in z-resolution. The most simple and commonly used method of opsin excitation involves the use of LEDs to illuminate an entire region of interest. This is frequently used for in vivo (Laxpati et al., 2014), ex vivo and in vitro modulation of neuronal activity (Klapoetke et al., 2014).
Recently, the development of more complex systems has resulted in the ability to excite optogenetic tools with increased spatial resolution. These systems vary greatly in complexity, with some using one-photon stimulation, some using two-photon stimulation and some integrable with either. Regarding two-photon stimulation, many systems effectively utilise femtosecond pulsed lasers to stimulate small three-dimensional volumes. However, as previously mentioned, two-photon stimulation of excitatory opsins has limitations in that the excited tissue volume may be insufficient to reliably generate a response. As a result, the stimulation volume is often moved in a circular manner to recruit activation of more channels in the membrane. This method has been found effective in the reliable generation of action potentials, however, the result is a restriction in the maximum temporal resolution achievable, which is dependent on the duration of the spiral.
Lastly, in recent years the use of computer-generated holography (CGH) with two-photon stimulation has allowed for stimulation with high spatial and temporal resolution, while also allowing multiple cells to be targeted simultaneously (Shemesh et al., 2017). Although, the CGH systems are very effective at achieving stimulation with exceptionally high resolution, their limitations come from their complexity and financial cost.
Other system considerations
Many other factors should be taken into consideration when performing an optogenetics experiment. Firstly, it’s important to ensure the light path of the excitation beam is clean and unobstructed. Additionally, if the system in use is aiming to achieve spatially precise stimulation, calibration of the light beam position should be undertaken before each experiment to ensure stimulation is occurring at the intended location. Moreover, if illumination is laser-mediated, the temperature of the room should be kept constant to ensure stability of equipment and minimise any changes to the spatial characteristics of the light beam due to expansion/contraction of any light-guiding components.
When stimulating for extended periods of time, many other considerations should be taken into account. Prolonged exposure to light can result in phototoxicity due to the release of reactive oxygen species from fluorophores (Icha et al., 2017). Optogenetic tools are usually tagged to particular fluorescent proteins and it is important to minimise excitation of both the optogenetic tool and the identifying fluorophore when searching for a cell of interest. Moreover, prolonged exposure to high intensity light can result in increases in the temperature of tissue which may alter physiological function and communication within the brain (Deng et al., 2014; Picot et al., 2018).
If using ionotropic opsins, which involve the rapid translocation of ions across the membrane, consideration of the influence of the alteration of ion concentrations is essential. For example, in the case of ArchT-mediated inhibition, the outward pumping of protons can result in local changes to extracellular pH (Ferenczi et al., 2016).
Optogenetic tools can be regarded as incredibly diverse and powerful instruments in probing communication within brain networks. When planning and executing an experiment using these tools, investigators should consider the many factors mentioned previously in order to construct a viable experimental paradigm.
Optogenetic Actuator | Output | Peak Excitation Wavelength | Kinetics | Spatial Targeting | References |
ChR2 | Cation influx leading to depolarisation | 470nm | Moderate | None | Boyden et al., 2005 |
ChR2 (H134R) | As for ChR2, with less desensitisation | 450nm | Slightly slower than ChR2 | None | Nagel et al., 2005 |
CoChR | Cation influx leading to depolarisation with highest photocurrent | 470nm
| Slightly slower than ChR2 | None | Klapoetke et al., 2014 |
SoCoChR | Cation influx leading to depolarisation | 470nm | Faster than CoChR | Soma-targeted | Shemesh et al., 2017 |
C1V1 | Cation influx leading to depolarisation | 550nm | Slightly slower than ChR2 | None | Yizhar et al., 2011 |
Chronos | Cation influx leading to depolarisation with very fast kinetics | 500nm | Fastest kinetics | None | Klapoetke et al., 2014 |
eNpHR3.0 (Halorhodopsin) | Cl- inward pump leading to cellular hyperpolarisation | 589nm | Moderate | None | Gradinaru et al., 2010; Chuong et al., 2014 |
Jaws | Cl- inward pump leading to cellular hyperpolarisation with high photocurrent: enables stimulation through skull | 632nm | Moderate | None | Chuong et al., 2014 |
(ArchT) Archaerhodopsin | H+ outward pump leading to cellular hyperpolarisation | 566nm | Moderate | None | Han et al., 2011; Chuong et al., 2014 |
GtACR2 | Cl- channel leading to prolonged cellular hyperpolarisation | 455nm | Fast | KV2.1 motif to target to somatodendritic compartment | Goverunova et al., 2015; Messier et al., 2018 |
OptoXR | GPCR activation depending on protein of interest (‘X’). Includes glutamatergic, adrenergic, dopaminergic and opioid receptors. | 473nm | Slow (G-protein activation) | None | Kim et al., 2017 |
Table 1: Commonly used optogenetic actuators and their properties
Other optogenetics articles
- Optogenetics: Shedding light on the brain's secrets
- #LabHacks: Choosing the best opsin for your optogenetics experiments
- BL-OG: Combining firefly physiology with optogenetics
- First human test of optogenetics highlights its clinical potential
- Optogenetics: Lighting the way for the future
- Top 10 discoveries in 10 years of optogenetics
References
Akerboom, J., Carreras Calderón, N., Tian, L., Wabnig, S., Prigge, M., Tolö, J., Gordus, A., Orger, M., Severi, K., Macklin, J., Patel, R., Pulver, S., Wardill, T., Fischer, E., Schüler, C., Chen, T., Sarkisyan, K., Marvin, J., Bargmann, C., Kim, D., Kügler, S., Lagnado, L., Hegemann, P., Gottschalk, A., Schreiter, E. and Looger, L. (2013). Genetically encoded calcium indicators for multi-color neural activity imaging and combination with optogenetics. Frontiers in Molecular Neuroscience. Volume 6, March, pp.1-29. doi: 10.3389/fnmol.2013.00002
Berndt, A., Schoenenberger, P., Mattis, J., Tye, K., Deisseroth, K. and Hegemann, P. (2011). High-efficiency channelrhodopsins for fast neuronal stimulation at low light levels. Proceedings of the National Academy of Sciences. Volume 108, Issue 18, pp.7595-7600. doi: 10.1073/pnas.1017210108
Boyden, E., Zhang, F., Bamberg, E., Nagel, G. and Deisseroth, K. (2005). Millisecond-timescale, genetically targeted optical control of neural activity. Nature Neuroscience. Vol. 8, Number 9, pp.1263. doi: 0.1038/nn1525
Cetin, A., Komai, S., Eliava, M., Seeburg, P. and Osten, P. (2007). Stereotaxic gene delivery in the rodent brain. Nature Protocols. Volume 1, Issue 6, pp.3166-3174. doi: 10.1038/nprot.2006.450
Chuong, A., Miri, M., Busskamp, V., Matthews, G., Acker, L., Sørensen, A., Young, A., Klapoetke, N., Henninger, M., Kodandaramaiah, S., Ogawa, M., Ramanlal, S., Bandler, R., Allen, B., Forest, C., Chow, B., Han, X., Lin, Y., Tye, K., Roska, B., Cardin, J. and Boyden, E. (2014). Noninvasive optical inhibition with a red-shifted microbial rhodopsin. Nature Neuroscience. Vol. 17, Issue 8, pp.1123-1129. doi: 10.1038/nn.3752
Deng, W., Goldys, E., Farnham, M. and Pilowsky, P. (2014). Optogenetics, the intersection between physics and neuroscience: light stimulation of neurons in physiological conditions. Am. J. Physiol. Regul. Integr. Comp. Physiol. 307, pp.1292–1302. doi: 10.1152/ajpregu.00072.2014
Ferenczi, E., Vierock, J., Atsuta-tsunoda, K., Tsunoda, S., Ramakrishnan, C., Gorini, C., Thompson, K., Lee, S., Berndt, A., Perry, C., Minniberger, S., Vogt, A., Mattis, J., Prakash, R., Delp, S., Deisseroth, K. and Hegemann, P. (2016). Optogenetic approaches addressing extracellular modulation of neural excitability. Scientific Reports. Volume 6, Article number: 23947, pp.1-20. doi: 10.1038/srep23947
Goverunova, E., Sineshchekov, O., Janz, R., Liu, X. and Spudich, J. (2015). Natural light-gated anion channels: A family of microbial rhodopsins for advanced optogenetics. Science. Vol. 349, Issue 6248, pp.647-650. doi: 10.1126/science.aaa7484
Gradinaru, V., Zhang, F., Ramakrishnan, C., Mattis, J., Prakash, R., Diester, I., Goshen, I., Thompson, K. and Deisseroth, K. (2010). Molecular and Cellular Approaches for Diversifying and Extending Optogenetics. Cell. 2010, 141(1), pp.154–165.
Han, X., Chow, B., Zhou, H., Klapoetke, N., Chuong, A., Rajimehr, R., Yang, A., Baratta, M., Winkle, J., Desimone, R. and Boyden E. (2011). A high-light sensitivity optical neural silencer: development and application to optogenetic control of non-human primate cortex. Front Syst Neurosci. 2011 Apr 13;5:18. doi: 10.3389/fnsys.2011.00018.
Hochbaum, D., Zhao, Y., Farhi, S., Klapoetke, N., Werley, C., Kapoor, V., Zou, P., Kralj, J., MacLaurin, D., Smedemark-Margulies, N., Saulnier, J., Boulting, G., Straub, C., Cho, Y., Melkonian, M., Wong, G., Harrison, D., Murthy, V., Sabatini, B., Boyden, E., Campbell, R. and Cohen, A. (2014). All-optical electrophysiology in mammalian neurons using engineered microbial rhodopsins. Nature Methods. Volume 11, Issue 8, pp.825-833. doi: 10.1038/nmeth.3000
Icha, J., Weber, M., Waters, J. and Norden, C. (2017). Phototoxicity in live fluorescence microscopy, and how to avoid it. Bioessays. 39, 8, 1700003, pp.1-15. doi:10.1002/bies.201700003
Kara, D. and Dahm, R. (2010). Transfection Techniques for Neuronal Cells. Journal of Neuroscience. Volume 30, Issue 18, pp.6171-6177. doi:10.1523/JNEUROSCI.0183-10.2010
Kim, C., Adhikari, A. and Deisseroth, K. (2017). Integration of optogenetics with complementary methodologies in systems neuroscience. Nature Reviews Neuroscience. Volume 18, pp.222–235. doi: 10.1038/nrn.2017.15
Kim, J., Hwa, J., Garriga, P., Reeves, P., RajBhandary., U. and Khorana, H. (2005). Light-Driven Activation of β2-Adrenergic Receptor Signaling by a Chimeric Rhodopsin Containing the β-Adrenergic Receptor Cytoplasmic Loops. Biochemistry. Vol. 44, No. 7, pp.2284-2292. doi: 10.1021/bi048328i
Klapoetke, N., Murata, Y., Kim, S., Pulver, S., Birdsey-Benson, A., Cho, Y., Morimoto, T., Chuong, A., Carpenter, E., Tian, Z., Wang, J., Xie, Y., Yan, Z., Zhang, Y., Chow, B., Surek, B., Melkonian, M., Jayaraman, V., Constantine-Paton, M., Wong, G. and Boyden, E. (2014). Independent optical excitation of distinct neural populations. Nat Methods. 11(3):338-46. doi: 10.1038/nmeth.2836.
Laxpati, N., Mahmoudi, B., Gutekunst, C., Jonathan, P., Zeller-townson, R. and Gross, R. (2014). Real-time in vivo optogenetic neuromodulation and multielectrode electrophysiologic recording with NeuroRighter. Frontiers in Neuroengineering. Volume 7, Article 40, pp.1-15. doi: 10.3389/fneng.2014.00040
Lin, J., Knutsen, P., Muller, A., Kleinfeld, D. and Tsien, R. (2013). ReaChR: A red-shifted variant of channelrhodopsin enables deep transcranial optogenetic excitation. Nat Neurosci. 2013 Oct; 16(10), pp.1499–1508. doi: 10.1038/nn.3502
Mantoan Ritter, L., Macdonald, D., Ritter, G., Escors, D., Chiara, F., Cariboni, A., Schorge, S., Kullmann, D. and Collins, M. (2016). Lentiviral expression of GAD67 and CCK promoter-driven opsins to target interneurons in vitro and in vivo. The Journal of Gene Medicine. 18(1). doi: 10.1002/jgm.2873
Marvin, J., Borghuis, B., Tian, L., Cichon, J., Harnett, M., Akerboom, J., Gordus, A., Renninger, S., Chen, T., Bargmann, C., Orger, M., Schreiter, E., Demb, J., Gan, W., Hires, S. and Looger, L. (2013). An optimized fluorescent probe for visualizing glutamate neurotransmission. Nature Methods. Volume 10, Issue 2, pp.162-165. doi:10.1038/NMETH.2333
Messier, J., Chen, H., Cai, Z. and Xue, M. (2018). Targeting light-gated chloride channels to neuronal somatodendritic domain reduces their excitatory effect in the axon. eLIFE. 2018;7:e38506. doi: 10.7554/eLife.38506.001
Miyashita, T., Shao, Y., Chung, J., Pourzia, O. and Feldman, D. (2013). Long-term channelrhodopsin-2 (ChR2) expression can induce abnormal axonal morphology and targeting in cerebral cortex. Frontiers in Neural Circuits. Vol. 7, January, pp.1-10. doi: 10.3389/fncir.2013.00008
Nagel, G., Brauner, M., Liewald, J., Adeishvili, N., Bamberg, E and Gottschalk, A. (2005). Light Activation of Channelrhodopsin-2 in Excitable Cells of Caenorhabditis elegans Triggers Rapid Behavioral Responses. Current Biology. Vol. 15, pp.2279–2284. doi: 10.1016/j.cub.2005.11.032
Naldini, L., Blomer, U., Gage, F., Tronot, D. and Verma, I. (1996). Efficient transfer, integration, and sustained long-term expression of the transgene in adult rat brains injected with a lentiviral vector. Proceedings of the National Academy of Sciences. Volume 93, pp.11382-11388. doi: 10.1073/pnas.93.21.11382
Naso, M., Tomkowicz, B., Perry, W. and Strohl, W. (2017). Adeno-Associated Virus ( AAV ) as a Vector for Gene Therapy. BioDrugs. Volume 31, Issue 4, pp.315-332. doi: 10.1007/s40259-017-0234-5
Perea, G., Yang, A., Boyden, E. and Sur, M. (2014). Optogenetic astrocyte activation modulates response selectivity of visual cortex neurons in vivo. Nature Communications. 2014(5), pp. 1-12. doi: 10.1038/ncomms4262
Picot, A., Dominguez, S., Liu, C., Chen, W., Tanese, D., Ronzitti, E., Berto, P., Papagiakoumou, E., Oron, D, Tessier, G., Forget, B. and Emiliani, V. (2018). Temperature Rise under Two-Photon Optogenetic Brain Stimulation Article Temperature Rise under Two-Photon Optogenetic Brain Stimulation. Cell Reports. Volume 24, Issue 5, pp.1243-1253. doi:10.1016/j.celrep.2018.06.119
Shemesh, O., Tanese, D., Zampini, V., Linghu, C., Piatkevich, K., Ronzitti, E., Papagiakoumou, E., Boyden, E. and Emiliani, V. (2017). Temporally precise single-cell-resolution optogenetics. Nature Neuroscience. Volume 20, Issue 12, pp.1796-1806. doi:10.1038/s41593-017-0018-8
Yamawaki, N., Suter, B., Wickersham, I. and Shepherd, G. (2016). Combining Optogenetics and Electrophysiology to Analyze Projection Neuron Circuits. Cold Spring Harb. Protoc. pp.840-847. doi: 10.1101/pdb.prot090084
Yizhar, O., Fenno, L., Prigge, M., Schneider, F., Davidson, T., Shea, D., Sohal, V., Goshen, I., Finkelstein, J., Paz, J., Stehfest, K., Fudim, R., Ramakrishnan, C., Huguenard, J., Hegemann, P. and Deisseroth, K. (2011). Neocortical excitation / inhibition balance in information processing and social dysfunction. Nature. Vol. 477, Issue 7363, pp.171-178. doi: 10.1038/nature10360
Sign up to receive our latest news
Find out about Scientifica's latest product releases, company news, and developments through a range of news articles, customer interviews and product demonstration videos.