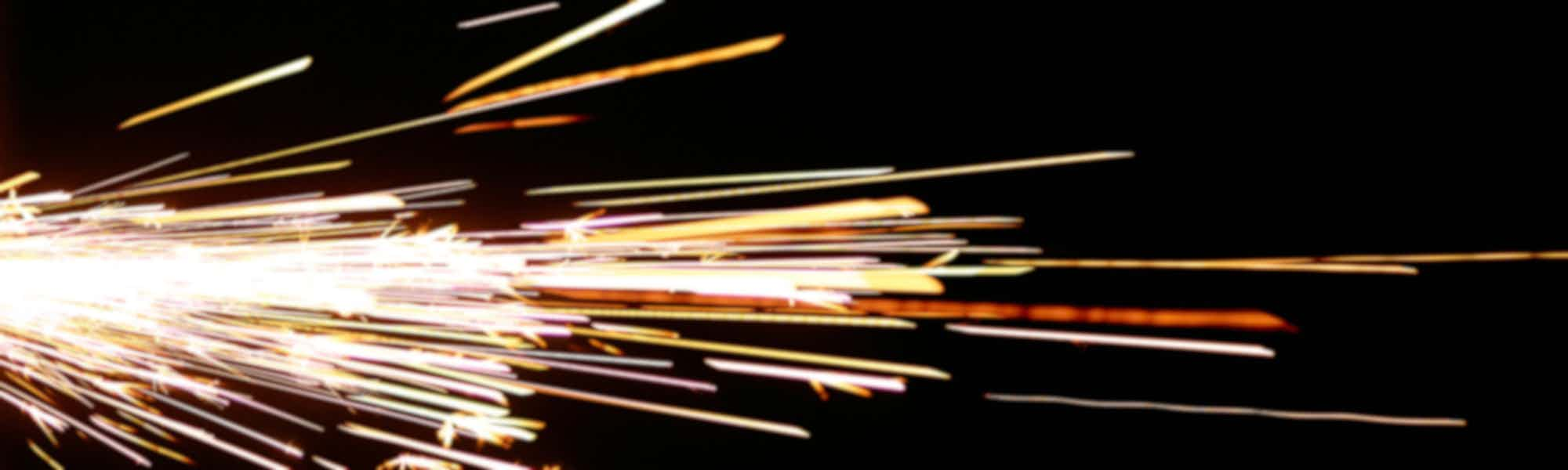
Multidimensional multiphoton imaging
By Tom Longden
Multiphoton imaging applies a high power, rapidly pulsed, long wavelength laser to generate planar excitation of fluorophores of interest. The basic physical principle that underpins this imaging approach relies on the fact that if two photons of a near-infrared wavelength arrive at a fluorophore within a vanishingly short window of time (in the range of one attosecond), they will correspond to an energy quantum that is equivalent to one photon of half their wavelength. This energy packet is capable of exciting the fluorophore from its ground state, causing it to fluoresce. Multiphoton imaging applies femtosecond pulsed lasers to generate this two-photon effect in biological samples, to limit excitation to fluorophores at the lasers point of focus. This effectively produces a confocal effect, as photon density in the planes above or below the point of focus is insufficient for multiphoton excitation. As a consequence, over- or underlying tissue is not excited and thus does not contribute to the resulting signal. By raster scanning the laser, high definition fluorescent images can be obtained.
Multiphoton microscopes take advantage of this effect to enable live imaging in thick tissue sections. Indeed, for imaging in complex tissues, such as the brain, multiphoton laser scanning microscopy offers many advantages over conventional confocal imaging. Firstly, the longer wavelengths used (typically 800 nm and above) allow for superior penetration of photons into the sample, enabling imaging at depth. Indeed, this modality has been used to visualize hippocampal neurons all the way through the intact overlying cortex (click here for an example). Secondly, by only exciting fluorophores at the point of the laser’s focus, photobleaching is dramatically reduced (a problem that has long plagued conventional confocal microscopy). Thirdly, infrared wavelengths cause much less photodamage than conventional single photon wavelengths, preserving preparation viability. Combined, these advantages enable performance of superior three-dimensional imaging in deep tissues when compared with many other microscopy approaches.
Previous generations of multiphoton microscopes have, however, been relatively slow at acquiring information in single planes and even more so in three dimensions, which limited their practical applications to only relatively unhurried biological processes that can be accurately sampled at low frequency. Observing fast intracellular events such as short-duration calcium signals (which can occur and resolve within milliseconds) or rapidly moving objects like red blood cells has thus been traditionally beyond the grasp of multiphoton microscopists, unless spatially restricted approaches such as line scanning are applied. Indeed, the relative slowness of galvo mirrors and motorized z drives kept the imaging frequency for significant volumes of tissue to <0.5 Hz. Modern multiphoton microscopes offer solutions to this issue and make acquisition of rapid volumes a reality. By combining resonant scanning mirrors to enable planar acquisition at 30+ Hz with piezo-actuated z-drives it is now possible to acquire volumes up to 100 µm in z-depth at several Hz. If the signal to noise ratio is high, dropping pixel resolution and dwell times can lend further improvements in acquisition rate (indeed, we have achieved ~8 Hz imaging 25 planes through 50 µm z at 256 x 256 resolution).
Resonant scanners have superseded traditional raster-scanning x/y-galvanometer mirror approaches, to enable fast acquisition in x and y dimensions. Typically, one galvanometer is replaced by a resonant scanning mirror that vibrates at a high, fixed frequency (>4 kHz) which enables much faster scanning of the plane of interest. To improve the speed of z acquisition, resonant scanning is combined with a piezo drive in which a voltage is applied to a piezoelectric crystal which responds with a uniform and consistent distortion, used to drive physical movement of the objective through the z axis at very high speeds.
Thus, this confluence of technologies makes it now possible to acquire volumes at physiologically relevant rates to permit four-dimensional physiological explorations. Simultaneous imaging of multiple fluorochromes further increases imaging dimensionality to permit multidimensional tracking of several processes at once (e.g. cell movement and calcium in x, y, and z over time). Our group is taking advantage of these developments to push our experiments imaging brain blood flow and its relationship to calcium signaling in the vascular endothelium and smooth muscle to the next level. As the brains vascular network is highly tortuous, one way to directly observe how blood flow through it is regulated is through rapid volume imaging. Resonant scanning with multiphoton lasers thus offers an elegant solution to this problem, and will continue to enable exciting discoveries in many aspects of physiology.
About the author
Tom Longden is an Assistant Professor in the department of physiology at the University of Maryland Baltimore. You can find more out about his groups work at www.longdenlab.org.
Are you interested in writing a Neurowire blog post?
Please email [email protected] for more information. Read examples of other guest blog posts here.
Sign up to receive our latest news
Find out about Scientifica's latest product releases, company news, and developments through a range of news articles, customer interviews and product demonstration videos.